Virtual Cocaine Lab
BOOK 2: Dopamine and Cocaine
Paul Garris: Primary Author
A dopamine neuron is a neuron that uses the neurotransmitter dopamine
for chemical neurotransmission. As will be discussed, dopamine neurons are
important for motor control, motivated behavior, and in mediating the effects
of drugs of abuse such as cocaine.
By binding to
receptors, neurotransmitters act as a chemical messenger or link connecting the
action potential from one neuron with a membrane potential in another. But
unlike receptors found in "classic" chemical neurotransmission, dopamine
receptors function a bit differently. In "classic" chemical neurotransmission,
neurotransmitter binds to receptors that open ion channels. This allows ions to
pass through the neuronal membrane. In this way, the chemical signal, the
neurotransmitter, is transduced into an
electrical signal, because ion flow will generate a change in voltage at the
postsynaptic membrane. If this voltage change reaches threshold, the target
neuron will fire an action potential. In contrast, the dopamine receptor is
called a G-protein coupled receptor.
Instead of directly opening an ion channel, dopamine binding to its receptor
activates a G-protein that in turn activates a second messenger inside the
target neuron. The second message can cause several changes in the postsynaptic
neuron. These changes include opening and closing ion channels, but they also
include gene transcription (the
synthesis of RNA from DNA) and protein synthesis (the translation of RNA into amino-acids sequences
to form proteins).
Chemical
neurotransmission is terminated by removal of neurotransmitter from the cleft.
For dopamine, like most neurotransmitters, this is done through transporter proteins
on the presynaptic neuron. Once inside the neuron, dopamine is either
re-packaged into vesicles for use again or degraded via degradative enzymes.
These enzymes maintain intracellular levels of dopamine at safe levels. Interestingly,
high concentrations of dopamine appear to be toxic, so only by degrading dopamine
or repackaging it into vesicles is the dopamine neuron protected. Also, similar
to the receptors found in the postsynaptic neuron, the presynaptic dopamine
neuron contains dopamine receptors itself. These autoreceptors function as a "thermostat," either shutting down
the dopamine neuron when it is too active or speeding it up when too lethargic.
It is worth noting that the synapse
between a dopamine neuron and its target appears to function rather differently
than in the "classic" view. In the "classic" view of chemical
neurotransmission, because transporters or degradative enzymes prevent
neurotransmitter from escaping the synaptic cleft, the action of
neurotransmitter is confined to the synaptic cleft. But at a dopamine chemical
synapse however, dopamine released into the cleft readily diffuses out. For
this reason, dopamine is often called an extrasynaptic messenger. Unlike "classic" chemical neurotransmission, the
target of dopamine release is not restricted to the postsynaptic
neuron. Instead, the target is any
neuron with a dopamine receptor close enough to the dopamine synapse to be
exposed to an effective dopamine concentration. Hence, in addition to "classic"
chemical synapses at their terminals, dopamine neurons also form en passant
("in passing") synapses. These synapses allow a dopamine neuron to affect a
target neuron without terminating the axon. The ability of dopamine to escape
the synapse readily is why this neurotransmitter can be measured chemically in
the brain without a sensor or probe that is small enough to be placed inside
the synaptic cleft. To date, no such probe or sensor exists.
Ch.
2: Dopamine neuron systems
In addition to extensive overlap
in brain anatomy, organization, and function, rats and humans also share
similar dopamine neuron systems. A neuronal system describes the origins, projections, and
terminations of a collection of like neurons. Thus, a dopamine neuron system is
defined by the incoming or afferent
neurons, the locations of dendrites, cell bodies, axons and terminals, and
finally the outgoing or efferent
neurons.
The brain
contains several dopamine neuron systems.
One important group originates in the hypothalamus. Consistent with the
function of the hypothalamus, these dopamine neurons are involved in sexual
behavior and the regulation of the pituitary gland. Although many of these
dopamine neurons both begin and terminate within the hypothalamus, others
project to and terminate in the spinal cord. This later subset of hypothalamic
dopamine neurons would be said to be descending in anatomical terms, going from the forebrain to the
spinal cord.
Another
important group of dopamine neurons originates in the midbrain. These dopamine
neurons are ascending because they
project to and terminate in the forebrain. The ascending dopamine neurons
originate in two regions of the midbrain, the substantia nigra and ventral tegmental area (see Figure 1).
Fig. 1
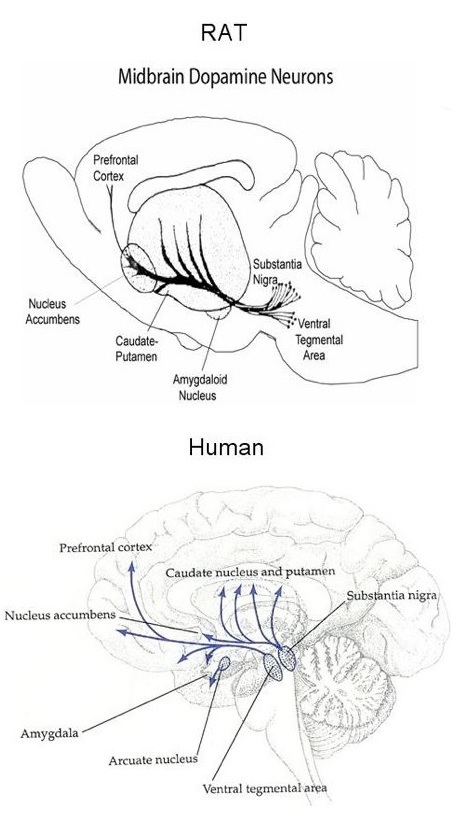
The brain is
symmetrical in rats and humans, so each hemisphere contains a substantia nigra
and striatum. The dopamine neurons originating in the substantia nigra
terminate in the striatum. The striatum is a part of the brain located
"under" the cerebral cortex. It receives projections from most, if
not all, cortical areas. Because the striatum is an important region of
the basal ganglia of the cerebrum, these dopamine neurons play an important
role in movement. Motor diseases such as Parkinson's
and Huntington's seriously affect the striatum.
Ch.
3: Approaches for assessing dopamine function
Several approaches have been used
to assess the role of dopamine in behavior (e.g., lesioning of dopamine
neurons, use of dopamine receptor drugs, etc.). This chapter will consider only
certain monitoring techniques.
One type of
monitoring approach is to use a microelectrode (a microscopic probe typically made of glass or metal) to record the firing
rate. The firing rate is the frequency of
action potentials a dopamine neuron generates over a period of time. This technique
is called electrophysiology.
Because the cell body is physically the largest portion of the neuron, it
generates the largest electrical signals. Hence, electrophysiological
recordings are usually performed in a region of the brain containing neuron cell
bodies. For midbrain dopamine neurons, this region would be either the
substantia nigra or ventral tegmental area. On the downside, to use
electrophysiology one must assume that an action potential occurring at the
cell body always causes dopamine release at the axon terminal. This may not
always be true. For instance, dopamine autoreceptors can regulate dopamine
release at the terminal independent of control by the cell body.
Other
monitoring techniques have been developed to measure dopamine directly in terminal
fields, which are those regions of the
brain where dopamine neurons make synapses (or "terminate"). For instance, the
striatum is the terminal field of midbrain dopamine neurons originating in the
substantia nigra. One widely used monitoring technique is microdialysis. In general, dialysis is a procedure in which some
but not all molecules move across a membrane. Molecules are typically excluded
based on size. Such a membrane is said to be semi-permeable. Based on this principle, a dialysis machine is
used to filter the blood of patients with kidney problems.
In
microdialysis of the brain, a probe is implanted in a terminal field so
dopamine can pass from extracellular fluid across a dialysis membrane and into
the center of the probe. By pumping artificial extracellular fluid through the
inside of the probe, dopamine is collected and measured outside of the animal
using very sensitive and selective instrumentation.
Although
microdialysis is used in animal experiments of many kinds, even to deliver
drugs to specific brain regions via a procedure called reverse dialysis, it has
two main disadvantages. First, samples are usually collected every few minutes.
This is slow relative to many behaviors. Second, microdialysis probes are
relatively large, about 300 microns (1000 microns = 1 millimeter) in diameter.
Thus, a probe can cause damage to the region where dopamine is monitored.
Another
technique for directly monitoring dopamine in terminal fields uses a chemical
microsensor. A common type is made from a
carbon fiber. Carbon is a biologically inert chemical, so it causes a minimal
reaction when implanted in the brain. And carbon fibers can be made very
small, 5 microns, which is 60
times smaller than the diameter of a microdialysis probe. Hence, a carbon-fiber
microelectrode causes less damage than a microdialysis probe. The carbon fiber
also provides an excellent surface for electrochemistry (the transfer of electrons between molecules), which
is how chemical microsensors measure dopamine. Two events must occur before
dopamine is monitored by a chemical microsensor. First, dopamine must come in
contact with the carbon fiber, or at least within a few nanometers. Second, to
pull off electrons from dopamine, the carbon fiber must be made positive electrically,
just like the positive end of a battery. Electrons are small charged particles
found in all molecules. The removal of electrons from a chemical is called oxidation. The rate of electrons flowing to the carbon fiber
during oxidation is related to the concentration of dopamine near the
microsensor. Consequently, monitoring dopamine using a chemical microsensor is
called electrochemical measurement.
In addition to
small size, electrochemical microsensors also have the important advantage of
making dopamine measurements very quickly, even several times a second. Thus,
chemical microsensors are a powerful tool for measuring dopamine changes during
behavior. The downside is that many chemicals besides dopamine can be oxidized.
This means that knowing what is measured by the chemical microsensor is an
important consideration.
Ch.
4: Dopamine neurons and motivated behavior
Motivated behavior
Motivated behavior is behavior
directed toward receiving a reward or goal. The reward may be natural (e.g.,
food, sex, etc.) or artificial (e.g., drugs of abuse). There are two components
or phases of motivated behavior. The appetitive phase consists of those behaviors related to
"approaching" the goal. In sexual behavior, for instance, the appetitive phase
consists of behaviors that establish, maintain, or promote sexual interaction.
Generally speaking, appetitive behaviors allow an animal to come into contact
with its goal. The consummatory phase represents the actual "consuming" of the goal. In the case of sexual
behavior, the consummatory phase is sexual intercourse. Collectively,
appetitive and consummatory aspects characterize a sexual encounter, which is a
motivated behavior. Addicted behavior is motivated behavior too.
The
neurobiology of motivation is a field that seeks to identify the neural
substrates (the brain regions, neuronal systems, neurotransmitters, receptors,
etc.) that mediate motivated behavior. As described below, the classic
experiment of intracranial self-stimulation demonstrated the existence of a brain reward system. The nucleus accumbens plays a central role in this system. Through
dopamine neurons, it links motivational information processed in the cortex
with emotional information processed in the limbic system, and then sends this
combined information to regions of the brain controlling motor output, hormone
release, and the fight-or-flight response. Thus, dopamine neurons terminating
in the nucleus accumbens play an important role in motivated behavior. Not
unexpectedly, the activity of these dopamine neurons changes during motivated
behavior. And such changes can be monitored while an animal engages in
motivated behavior.
Intracranial self-stimulation
One of the earliest experiments
identifying a relationship between nucleus accumbens dopamine neurons and
motivated behavior was intracranial self-stimulation. During this experiment, a stimulating electrode is
implanted in the ventral tegmental area to activate dopamine neurons
artificially using electrical pulses. The stimulating electrode and the
instrument generating the electrical pulses are connected to the lever of a bar
press machine. When the animal presses the lever, electrical pulses are
delivered to the stimulating electrode. Thus, the animal controls stimulation
of its dopamine neurons. (This type of control is called contingent. When a scientist controls the stimulation, the
control is called non-contingent.)
To obtain the "rewarding" electrical stimulation, rats lever press at
astonishing rates, sometimes as fast a five times per second. They will also
lever press continuously for hours.
Early studies
with intracranial self-stimulation were very informative. Indeed, the highest
rates of lever pressing during intracranial self-stimulation occurred with the
stimulating electrode activated dopamine neurons directly. Collectively, such
experiments led neuroscientists studying the neurobiology of motivated behavior
to conclude that dopamine was the neural substrate of reward. In this view,
dopamine is released when the animal consumes the reward, and the amplitude of
dopamine release reflects the magnitude of the reward (or how good does this
reward make it feel). Thus, dopamine is said to act as the neural substrate
of reward during the consummatory phase of motivated behavior. Moreover, all rewards, whether natural (e.g.,
food, sex, etc) or artificial (e.g., electrical stimulation or drugs of abuse),
were thought to be mediated by dopamine release.
Recent results
challenge the traditional view that dopamine is only the neural substrate of reward.
One of the key considerations with this new evidence is this: To understand
fully the role of dopamine in motivated behavior, one must be able to monitor
dopamine very quickly because behavior can be very fast as well. For example,
microdialysis clearly shows that dopamine release increases when animals lever
press for a rewarding electrical stimulation. But rats will bar press at rates
upwards of 5 per second during intracranial self-stimulation. Microdialysis can
in no way tell us what happens to dopamine release with each bar press. Nor can
it tell us what happens to dopamine levels just before the animal bar presses.
Both the bar press and the time leading up to it constitute the appetitive
phase of this motivated behavior. Because of faster sampling rates, chemical
microsensors can do both these things.
One type of chemical microsensor technique, fast-scan cyclic voltammetry
(or voltammetry), can measure dopamine
10 times per second.
When voltammetry was used to monitor dopamine release, some very
unusual findings were obtained. For example, when the electrical stimulus was
applied by the experimenter, the same stimulus that animals will lever press
for, dopamine levels increase in the nucleus accumbens. This result suggested
that each lever press during intracranial self-stimulation appeared to be
rewarding in the same way as other rewarding stimuli. During
training of lever press behavior, when the animal learns to associate the lever
with the rewarding electrical stimulation, voltammetry showed that dopamine is
also released during intracranial self-stimulation. However, in well trained
animals, intracranial self-stimulation did not release dopamine; that is,
animals lever pressed and received electrical stimulation but dopamine release
did not increase.
Moreover, the
same record of lever press activity, when replayed to the same and other
animals, caused dopamine release. Remarkably, these animals received the same
number and timing of the electrical stimulation as during intracranial self-stimulation,
but in this case, dopamine release was observed. Remarkably, non-contingent but
not contingent electrical stimulation caused dopamine release. What this
interesting experiment demonstrates is that dopamine is not absolutely
necessary for the consumption of an award. Instead, it appears to play a role
perhaps related to learning of the cues associated with reward. In intracranial
self-stimulation, the cue would be the lever press. This type of learning is
called associative learning.
Ch.
5: Dopamine neurons and drugs of abuse
In the United States, one of the
more prominent drugs of abuse is cocaine.
Cocaine has multiple effects on the body, both peripherally outside the brain
and centrally within the brain. Cocaine was originally used in medicine as a
local anesthetic during eye surgery. The effect as a local anesthetic is
independent of any action cocaine has on the brain. Cocaine also has potent
effects directly on the cardiovascular system, which consists of the heart and
all of the blood vessels. In general, cocaine increases blood pressure by
stimulating the beating of the heart and by causing blood vessels to constrict.
Large doses of cocaine can result in cardiac failure. The actions of cocaine on
the brain are generally thought to be mediated by three neurotransmitters:
dopamine, norepinephrine, and serotonin.
Two classic
experiments are used to demonstrate that cocaine and other drugs of abuse are
rewarding: conditioned place preference
and drug self-administration. In
conditioned place preference, an animal is released into a chamber that is
demarcated into different quadrants. Over a period of time, the animal, when
venturing into a specific quadrant, is injected with the test substance. After
sufficient time, the animal learns the association between a quadrant and the
drug injection. The animal is then allowed to enter the chamber but without any
drug injection. If a drug is considered rewarding, the animal will tend to
localize in the quadrant causing the drug injection. If a drug is considered aversive, the animal will tend to localize in the other
quadrants. If a drug is considered neutral, no pattern of localization will
occur.
Drug
self-administration is analogous to
intracranial self-stimulation, except that instead of a lever press delivering
a rewarding electrical stimulation, an injection of a drug is administered. The
drug is typically delivered intravenously. The goal of cocaine
self-administration training is to teach a
laboratory rat to press a lever in order to obtain an injection of cocaine.
Cocaine will be injected into the jugular vein by a cannula, a small hollow
tube inserted into the blood vessel. The jugular vein will carry the cocaine to
the heart, which will then pump it to the brain in a matter of 30
seconds. Lever pressing rates for drug
self-administration are considerably lower than for intracranial
self-stimulation or even lever pressing for food reward. The reason for this is
that drugs have a long period when they are active in the brain, so they do not
need to be administered as often.
Two regimens
are used to train animals to self-administer drugs: manual shaping and autoshaping. In manual shaping, the experimenter guides the
animal to lever press for the drug using the technique of successive approximation.
In other words, the researcher reinforces behavior increasingly similar to the
wanted behavior. In contrast, autoshaping lets chance do most of the work. Both
manual shaping and autoshaping are time-consuming processes, often taking
several days to train an animal to self-administer a drug.
The
pharmacological effect of cocaine on dopamine neurons is that cocaine blocks
the dopamine transporter. This prevents dopamine from being transported back
into the presynaptic neuron from the synaptic cleft. Norepinephrine neurons and
serotonin neurons have their own specific transporters too. Cocaine binds to
and blocks the action of the norepinephrine and serotonin transporters as well.
Thus, by preventing the removal of released neurotransmitter, cocaine increases
the extracellular levels of dopamine, norepinephrine, and serotonin in the
brain.